Fossil fuels have a great impact on production costs, production prices, and the environment. Reserves of non-renewable sources are sharply decreasing. Fuel oil has reserves for next 50.6 years on global production. These predictions have been made by British Petroleum in June 2016 [1]. Therefore, many international regulations and strategies have become obligatory for all countries. These international regulations and strategies suggest the use of fuel from renewable sources (green energy). There is a tendency to increase the use of renewable energy such as solar energy, energy from water, wind, biomass and geothermal energy. The world’s total energy supply in 2017 compared to the past decade shows the greatest reduction in oil utilisation [2]. Coal supply is slightly decreased, but there is growth in the use of natural gas. Increasing the use of renewable energy sources has a positive trend. This is very important for the following benefits:
Smaller emission of CO2;
Use of cheap energy;
Decrease in the production cost and the price of the product.
Solar thermal energy is widely used in many processes to reduce the heat demand. The Sun is a renewable source of free thermal energy.
The International Energy Agency (IEA) continuously analyses the use of different types of energies and their demand in the world. Based on their report [3], global solar thermal capacity in active facilities, as well as global solar energy yield, has risen rapidly over the past decade. They determined the need of heat for different types of industrial branches as total heat demand for low and medium applications in Solar Heat Integration in Processes (SHIP). The chemical industry, together with the food-processing industry has the main solar heat applications. This number of applications in production processes basically depends on the temperatures of utilities. Kalogirou [4] has made an important classification of temperature ranges in industries for specific processes where solar thermal energy can be used.
However, the use of solar heat has a disadvantage. The heat cannot be stored for a long time. Generally speaking, the use of solar thermal energy depends on the heat storage systems, weather conditions and insolation of the region.
Many authors reviewed the use of solar thermal energy. But most of them analysed applications, projects and solar thermal systems in specific production systems or in particular country or region. Pirasteh et al. [5] made an overview of the use of solar energy in the drying process in industrial systems. Systems with different solar collectors have different efficiencies. They analysed the possibility of heating dry air for consuming in a dryer. All these are compared on the basis of economic, environmental and political aspects. Furthermore, Farjana et al. [6], [7] found the main SHIP projects that exist in Australia and compared with the situation in India, Germany, Austria, USA, Spain, China, South Africa, Mexico, France and Greece. In the same comparison are given the most developed industrial sectors that have a lack of renewables. Similarly, Jia et al. [8] made an analysis of the status of solar heat used in Chinese industry. They reviewed the use of solar heat integration through values of factors in the following areas of industry: tobacco, food processing, paper, textiles, chemicals, wood processing, medical, plastic and rubber, oil/crude oil and gas exploitation, industrial sectors for fabricated metal products. The situation in Chile, Jordan, Italy, Brazil, and Malaysia has also been reported. They indicate the existence of great potential in the food industry and the chemical industry.
Polygeneration is perhaps the most interesting process these days. Murugan and Horak [9] found combinations used for tri- and polygeneration. These combinations include the use of solar energy, such as: Combined Cooling, Heating and Power generation (CCHP), Photovoltaic – Thermal collectors (CPVT), and solar-fossil fuel hybrid system. They mention the use of mathematical programming to optimise systems that include a variety of energy sources. In addition, Jamil et al. [10] made a comprehensive overview of the technologies used for renewables in UAE. Other types of renewables include solar heating systems. This includes solar heat concentration (so-called Solar Islands) and central heating of the water as a hot water distribution system. Yadav and Banerjee [11] examined the solar thermal systems for thermochemical purposes in industry. Production processes that require high-temperature heat can be provided from solar concentrators. The heating of reactors is directly supported by such solar heating systems with concentrators. The solar heat plants in Chile are part of the research done by Grageda et al. [12]. Most of them are plants for electricity generation. ORC is very attractive technology for conversion of waste heat into electricity. Zhai et al. [13] analysed the use of heat generated by ORC from renewables. Solar heat as renewable is a good source for electricity production in cogeneration systems. It is directly exchanged with evaporator of ORC. Different studies describe systems that use concentrators for direct supply of heat to ORC and the night-time period is covered by heat storage of high-temperature heat that supports continuous production of electricity. Moreover, heat supply can be a combination of different sources.
Klemeš et al. [14] mentioned the use of solar heat for process integration. They reviewed the development of Pinch technology. Solar heat integration with Pinch technology replaces the energy of fossil fuels in production up to 40% in different cases. There is another method used for the same purpose by Marinelli et al. [15]. They tried to explain the use of Life Cycle Thinking (LCT) as a method for making decisions in the design of residential heat pumps. That includes Life Cycle Costs (LCC), Life Cycle Assessment (LCA), Social-Life Cycle Assessment (S-LCA) and Life cycle environmental impact as complete opinion for the designed system. According to Marinelli et al. [15], renewables improve the sustainability of heat pumps.
Recently, units that combine photovoltaic panels and thermal solar collectors were designed. Brahim and Jemni [16] discussed about development of such modules as well as good economic performances in different areas of use. Additionally, Kannan and Vakeesan [17] analysed the use of solar energy with photovoltaics and thermal heat systems. They emphasise the use of photovoltaic. Wang et al. [18] reported about possibilities in use of solar radiation in the ammonia refinery. The system consumes solar heat, sunlight (for photo-reaction) and electricity generated from sunlight. They are directly integrated into the process.
The impact on the environment is also very important. Shahsavari and Akbari [19] determined the potential of solar energy in reducing Greenhouse Gases (GHG) emission. Emphasis is given to developing countries.
Most reviews refer to the use of solar heating systems and analyse a certain problem in the use or design of these systems for heat supply for production processes. There are case studies that are the basis of those reviews, but the design methodology is not given or it is just mentioned. The need to draw up a summary of the main techniques or methodologies used to integrate the heat of solar heating systems is the goal of preparation of this paper. The main task was to systematise the methodologies used to integrate solar heat in production systems. Also, finding links between them and standard procedures in the design of such plants.
The configuration of solar heating systems is very important for the storage and transfer of heat to the production system. In principle, it consists of solar modules, pipelines with pumps, and/or heat storage system.
The solar panels used to collect solar heat can be divided into two types as collectors and concentrators. The collectors have following constructions:
Flat-plate solar collectors;
Evacuated tube collectors;
Cylindrical solar collectors;
Conical solar collector;
Parabolic solar collectors;
Triangular shape solar collector.
Tchanche [20] made a classification of different types of solar heat collectors based on operating temperatures. Similarly, Farjana et al. [6] divided types of solar collectors according to the characteristics of operating temperature ranges and the ratio of the concentration of direct insolation. Furthermore, Lorenzini et al. [21] confirms the four main types of collectors that have the best ratio between costs and benefits applied under different conditions of use.
Moreover, Suresh and Rao [22] report about three categories of solar heat collectors as Low Temperature Collectors (LTC) (up to 80 °C), Medium Temperature Collectors (MTC) (for industrial use with temperature range between 80-400 °C) and High Temperature Collectors (HTC) (above 400 °C).
The disadvantages of using solar thermal energy are periodic insolation (day/night), weather conditions, and geographical location of the plant. The solution for these problems is the use of heat storage systems.
Heat storage systems are determined by different principles of heat accumulation by the material they contain. There are two main concepts for heat storage: sensible heat storage (with water, thermal oils, and solids), and latent heat storage (with phase change or chemical changes of material) [23]. These processes are based on the following [24]:
Sensible heat storage in saturated liquids at non-pressurised and pressurised liquids;
Sensible heat storage in solids;
Latent heat storage (high heat storage capacity per volume);
Thermochemical storage (high heat storage density, with low heat lost, not enough developed).
IEA [23] reported about importance of fluids used in industrial heat storage systems. The most widely used medium for heat storage is water, but there are some other fluids that can be used in these systems. Expenses for heat storage systems depend on the characteristics of media for heat storage (physicochemical), the heat capacity, the design of the reservoir, and allowed temperature changes [25]. Farjana et al. [6] described the selection of solar equipment needed for solar heat integration in the conventional systems. They determined following methods for selection:
Pre-heating of water for evaporation processes;
Integration on distribution level where high temperatures are required (steam) (there should be selected concentrating solar collectors);
Direct coupling with a specific process.
Heat storage can have a fixed or changeable volume. On the other hand, there is a storage with closed loop of storage medium that exchanges heat through internal or external heat exchangers. These storage systems can be connected in parallel or series to satisfy the heat demand of the company. Figure 1 shows different schemes of connections between collectors and heat storage tank or production process streams. Non-tracking collectors are fixed at one position. They have tubes inside modules that take solar heat and bring to the heat storage tank or production process. On the other hand, heat taking medium in tracking collectors (track maximal insolation position) is going through the absorber tube.
Solar heat accumulation systems can have different designs based on its purpose. There are systems with direct charging:
Systems with external heat exchangers and stratification valves;
System with external heat exchangers and mixed charging return flow;
System with internal heat exchangers;
Systems with heating lance.
The direct charging system (Figure 1a) is cheap and robust. The collector liquid is the heat storage medium. Systems with external heat exchangers and stratification valves (Figure 1b) has high loop efficiency. The role of the stratification valve is to control the inlet flow related to the temperature. The heat storage medium is different from the liquid in the solar collector. System flow to the external heat exchanger and mixing valve (Figure 1c) has fast process supply with high integration temperatures. Charging return flow is mixed to reach set charging temperature. Total volume is charged only if the load is covered. Heat storage has function as a hydraulic separator in case when process return flow has a stratified inlet. A more economical solution is a system with an internal coil tube heat exchanger (Figure 1d). It reduces heat loss from pipes and has costly heat exchanger. This system is designed for small-scale systems. System with heating lance is also for small solar heating systems (Figure 1e). It contains stratification devices. The system has good stratification, and it is expensive.
Storage charging strategies for SHIP: direct charging system (a); external heat exchanger system with stratification valve (b); external heat exchanger system with mixed charging return flow (c); internal heat exchanger system (not common for SHIP) (d) and heating lance system (not common for SHIP) (e) [23]
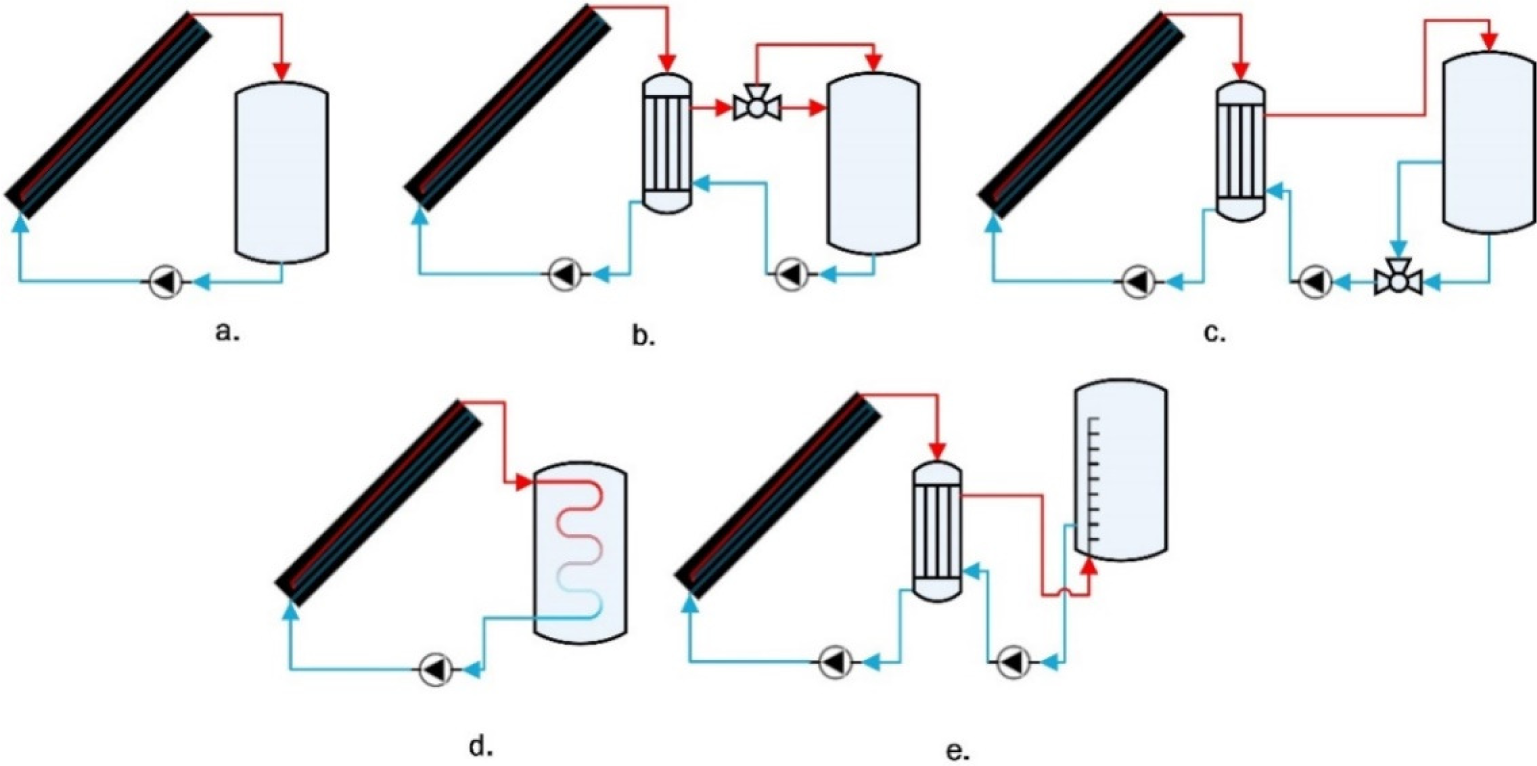
The heat storage materials vary in relation to the set storage temperature, or temperature range of the heat accumulation, as well as the physical and chemical characteristics of the storage medium. Berenguel et al. [24] proposed the use of mineral thermal oil for temperatures at 300 °C and silicon or synthetic oils at lower pressure for temperatures up to 410 °C, as an economical solution. Peng et al. [26] analysed the formation of nitrogen oxides from “solar salts” decomposition.
Nowadays, many researchers increase the efficiency of solar heating systems using nanofluids as a working medium. There are many benefits from nanofluids in solar heating systems [27]. The nanoparticles in nanofluids have small dimensions, but a large surface for heat exchange. The material of nanoparticles can have a high heat capacity. They can improve optic features such as lower emittance and higher absorption of solar heat. Thermal conductivity (heat transfer coefficient), high density and suspension stability are valuable properties of nanofluids. The specific heat capacity of nanofluids is determined with eq. (1) [28]. Its value depends on heat capacities of components in fluid (Cpbf) and nanoparticles (Cpnp), densities of base fluid (ρbf) and nanoparticles (ρnp) and the ratio of mixture (φ):
(1)
Otanicar et al. [29] compared different combinations of nanofluids. The use of graphite, silver, or other materials can increase the efficiency of solar heating system for approximately 5%. In addition, Yousefi et al. [30] studied the behaviour of aluminium oxide (Al2O3) – water nanofluids. The efficiency of solar collectors increased by 28.3% in case of 0.2% weight fractions of Al2O3. Other scientists have experimented with copper (Cu), cupric oxide (CuO), titanium dioxide (TiO2), as solid nanoparticles and have raised the efficiency of the solar heating system [31]. Salavati Meibodi et al. [32] analysed nanofluids prepared by silicon dioxide (SiO2) and ethylene glycol for the efficiency and performances of solar collectors. Similarly, He et al. [33] investigated the performances of solar collectors using Cu-H2O nanofluids. Faizal et al. [34] improved the energy, economic and environmental impact in use of metal oxides as part of nanofluids in heat transfer. The use of nanofluids in improvement of performances and efficiency of solar collectors is summarised by Elsheikh et al. [27].
Many projects for solar thermal integration are already being implemented in many countries. It is attractive in areas with high annual insolation. Every industry or human activity involves solar thermal energy use. Solar heating systems can be divided according to their way of connecting with the production processes or the complexity of the design. They can be directly connected without prior analysis of the production process, or after optimising with Pinch technology, mathematical modelling or other approaches.
The food processing industry uses solar heat systems the most intensively. The first applications were implemented in that industry. They are based on direct connection of the hot stream from the solar heating system with parts of production process. Quijera et al. [35], [36] analysed dairy production facility and fish canning processes. They made feasibility for integration of solar thermal energy with process streams for their heat demand. The solar heating system is connected to the hot water boiler that makes additional heating to the target temperature for milk production needs [36]. The heat pump is included in a similar project for canned fish factory [35]. Bühler et al. [37] optimised the use of solar thermal energy in German dairy production processes. Similarly, Allouhi et al. [38] inspected milk production factory in Casablanca. They tried to integrate solar thermal energy in all processes in the plant. All parts that require heat are taken into account for process integration. Milk chilling, fruit cleaning, milk pasteurisation and fruit drying are included in use of solar heat. Moreover, Wallerand et al. [39] analysed a dairy plant in Switzerland. Production of concentrated milk, yogurt and other dairy products is integrated with a combination of solar heating, photovoltaics and heat pumps.
The brewery industry invested in some solar heating projects. Mauthner et al. [40] investigated the use of low temperature solar heat in manufacturing of malt and beer. They determined the temperature intervals that exist and intervals that are needed in breweries. Mauthner et al. [40] analysed the malt and beer production processes in brewery “Goess” in Austria, brewery “Valencia” in Spain and malting plant “Vialonga” in Portugal (Heineken Group). Based on data they collected, Mauthner et al. [40] determined the percentage of heat demand that can be covered by solar heat. Similarly, Müller et al. [41] analysed the possibilities for solar heat accumulation in two German food companies: brewery AllgäuerBrauhaus AG and German dairies. Factors for solar heat usage in these types of companies depend on production technology. So, potentials for solar heat use are different. Müller et al. [41] find that breweries in Germany have lower heat demand than a dairy industry.
Extraction of essential oils from stored plants like Eucalyptus leaves, Peppermint leaves and Pinus for medical purposes is important to be done as soon as possible. Afzal et al. [42] developed a solar distillery equipment. They used it in solar heating for extraction of essential oils. Economic analysis of this case showed very low payback period. They concluded that the solar distillation is very successful project for use in agriculture.
There are some general studies for solar heat integrated systems in different areas of human living. Beath [43] reported about potential for use of solar heat as industrial energy sources in Australia. Insolation of industrial areas in Australia and the type of industry in those areas provided great opportunities for use of solar heat. That could decrease production costs, and it can minimise the use of fossil fuels. Fuller [44] made a similar study. He analysed already installed solar heating systems in different kinds of facilities in Australia. His analysis determined the thermal and economic performances of installed solar heating systems.
Textile industry also has heat demand. That is especially expressed in dyeing processes. Frey et al. [45] analysed solar heat installed in southern German textile industry. They calculated 81% primary energy savings in the production process. Similar to them, Carnevale et al. [46] made feasibility study for integration of the solar heating system in Italian textile industry. They integrated solar heating system with utility production unit (steam boiler). Textile industry in Morocco is one of the main branches of industry in that country. There is a great possibility for integration of solar heat within processes. Feasibility study about that is done by Calderoni et al. [47]. According to them, heat integration is not enough economically acceptable for implementation. Similar study is done for Benetton dyeing industry in Tunisia. Frein et al. [48] analysed solar thermal plant integrated to the process of dyeing in textile. The plant contains 1,000 m2 collectors. The water in the heat storage tank is heated through external heat exchanger. That system has set maximum and minimum allowed temperatures.
Many countries in North Africa and the Middle East have no reserve of drinkable water. They have possibilities for processing of sea water to get potable water. Solar thermal energy can help in this area, too. Bait and Si-Ameur [49] analysed a system for distillation of water for its purifying. That distillation is based on solar water vaporizer called “Solar still”. It contains a cylindrical glass inside which is positioned a copper coil tube for heating fluid. Directly connected solar heating systems can support preheating of water for production or steam generation (hot utility production). Joubert and Van Niekerk [50] made feasibility study to use the solar heating system for a company in Northern Cape. There is integrated solar heat system with steam boiler. It minimally can replace 20% of needs for heating water up to the boiling point. They calculated the possibilities for replacing more heat needed for the production system. Their study reported for replacing of 60% hot water demand with solar heat.
Generating steam (as utility) from solar thermal energy has become a reality. It is possible to directly generate steam at high temperatures, higher than 450 °C [51]. Prieto et al. [51] made a review about heat storage used for Direct Steam Generation (DSG) technology from solar thermal energy (Solar towers). This technology avoids intermediate liquid for heat transfer, reduces the cost and increases performances. DSG technology is already used in few power plants in the world. These plants are located in Southern Spain, South Africa (Khi Solar One) and the United States (Ivanpah Solar Project). Solar thermal energy can be accumulated in special devices. Some of these plants use superheated steam storage, but for a very short time of accumulation. Thermal Energy Storage (TES) systems for high temperatures and a longer time of accumulation, do not exist.
Brock et al. [52] suggested use of solar heat in Italian brick manufacture. They proposed solar heat field for concentration of thermal energy and generation of steam. The generated steam is directly connected to the production process via heat exchanger in the production system. Moreover, Buscemi et al. [53] suggested the use of heat storage made by concrete. The stored heat is transferred with water or oil to the pasta factory. The system generates superheated water (135 °C, 8 bar). It is used for pasta dryer and hot water generation. That has minimised the heat consumption in the factory by approx. 40%. Gil [54] explained possibilities for use of solar thermal energy in production of cork. The technology used for cork production (cork boiling process) uses hot water generated from solar panels.
Chemical industry can have benefits from solar heating systems, too. Xu et al. [55] analysed the use of solar heat in coal power plant. Namely, solar heat is used for drying of lignite and its pyrolysis. That decreases heat demand in preparing coal for steam boiler. Production of hydrogen can also be connected with use of solar thermal energy. Hoffman [56] made an outlook of hydrogen production with solar heat in South Africa. There are a few technologies for hydrogen production. Methane/steam reforming process uses heat. Steam can be generated from parabolic troughs. The solar heating system has closed loop with tanks for cold and hot fluid. Hot fluid is used for heating water through the heat exchanger. The heated water is used in generation of steam and reforming process.
IEA team coordinated by Bettina Muster prepared integration guide for solar integration with industrial processes [3]. They determined possible points for interaction between both systems. Those points are Integration on Supply Level (includes: water preheating for steam boilers, additional heating of Heat Supply Storage and Heat Supply Network) and Integration at Process Level (includes additional heating of Process Heat Storage, heating of processes and vessels, and heating of the process heating medium).
Liew et al. [57] examined the Total Site Heat Integration (TSHI) of renewable energy sources within production processes and urban systems. They reviewed different optimisation methodologies that can be used for TSHI projects. District heating is interesting for satisfying a part of its heat demand with solar thermal energy. Similarly, Joly et al. [58] inspected the solar heating system for use in the district heating system near Goteborg, Sweden. They used Task 52 from IEA. Their project is successfully realised through the combination of solar heating and wood pallets. Moreover, Eiholzer et al. [59] used Pinch technology for integration of solar heating systems in medium-sized Brewery in Scotland. They substitute utility stream with the solar heat stream.
Fluch et al. [60] reported about GREENFOODS project that involves many food and beverage companies (bakeries, breweries, dairies, fruit and vegetable processing and meat processing) in Austria, Germany, Poland, Spain and the UK. The aim of the project is increasing the energy efficiency for analysed types of companies and replacing the fossil fuel heat sources with renewables. Researchers in GREENFOODS identified the potential for energy efficiency and use of renewable energy sources like solar heat. They calculated 4,400 GWh energy savings and reduction of CO2 emission for 145,000 t per year.
Many authors report about the possibility of using different combinations for the generation of heat from the sun. They have suggested sun energy storage as electricity (photovoltaic). The stored electricity in batteries can be easily used for heating. Islam et al. [61] combined solar thermal energy with solar electricity, Reverse Osmosis (RO), hydrogen production and water purification processes. In case of low solar heat, they suggested using of Organic Rankine Cycle (ORC). Amoresano et al. [62] made a study for possible integration of solar thermal energy with the system of biomass energy plant. Both renewable energy sources are used in combination with steam generation and supply of ORC plant (small power plant). Similar projects with cogeneration systems are done by other researchers. Abdelhady et al. [63] analysed use of cogeneration system with solar heat for petrochemical industry. Similarly, Sedić [64] in his thesis combined solar heat integration and use of ORC for crude oil atmospheric distillation plant in Sisak refinery. Furthermore, Freeman et al. [65] analysed power plant based on solar heat integration and ORC. The heat storage of that system is the place where heat is exchanged between two separate systems, solar heating system and ORC. TES is used for accumulation of heat at high temperatures. ORC transforms heat into electricity. Moreover, Rahbar et al. [66] proposed new system for production of electricity. They proposed a system of solar concentrators with photovoltaics (CPV/T) connected to ORC systems. This system produces electricity with photovoltaic layer on solar collector ‒ concentrator. The reflected solar radiation heats the central tube with nanofluid flow. The working fluid is connected to ORC. There low-temperature heat is transformed into electricity. The whole system is designed with method of direct connection of solar heating system and ORC with aim to generate electricity for the production system. Similarly, Bellos and Tzivanidis [67] simulated and analysed trigeneration system that include solar heat system with heat storage, ORC and absorption heat pump. The absorption heat pump use lithium bromide water (LiBr-water) as working fluid. ORC works with organic fluid and the working fluid for solar heating system is thermal oil. All these parts have an important role for use and transformation of heat energy. The heat received in parabolic collectors is stored in heat storage tank. The accumulated heat is transferred to the ORC evaporator. ORC use that heat for production of electricity. Its condenser is connected with absorption heat pump. The absorption heat pump produces heat and cold simultaneously. All hot and cold sources can be connected with process streams. The described system is used for supply of electricity, as well as for heating and cooling.
Most articles that analyse ORC are connected to solar heating systems and the direct interaction between them with the main aim to produce electricity. This type of ORC is known as solar ORC. A similar system for solar ORC is analysed by Baccioli et al. [68]. They used solar heat system with parabolic collectors to supply ORC with heat. The other authors developed the basic model of small-scale solar ORC. Taccani et al. [69] analysed the prototype of solar ORC installed in Florence, Italy. They got poor performance in their system. The reason for that is low solar radiation and bad thermal insulation. They suppose that system will get better performance with higher solar radiation conditions. Patil et al. [70] tried to combine ORC with solar thermal energy and solar photovoltaics.
In another work of Bellos and Tzivanidis [71], they analysed the possibility of use of solar heat and waste heat with ORC system for production of electricity. Solar heating system stores heat into the reservoir for heat storage. Stream from heat storage as well as stream of waste heat enters the Heat Recovery System (HRS). HRS is actually a heat exchanger connected to ORC as its evaporator. The order of entrance of waste heat stream and solar heat stream depends on their temperatures. The first heating of ORC working fluid is done with streams that have lower temperature. Bellos and Tzivanidis [71] directly connected waste heat from production to the described system.
There is some research about cooling systems that work on a solar heating system. Sansaniwal et al. [72] made energy and exergy analysis of different systems that use solar heating. One of them is a refrigeration system based on using heat stored in TES for cooling purposes.
Integration of solar thermal energy in production processes is very interesting for ethanol distillation systems. Temperatures in alcohol distilleries are relatively low. That is one of the advantages of these facilities. Vargas-Bautista et al. [73] made a simulation of the distillation system for production of 95%wt. ethanol in Monterrey, Mexico. They used evacuated tube solar collectors and parabolic trough. The simulation is made with TRNSYS software. They found very high energy savings, especially in case of use of parabolic trough. The investment is very profitable for the company.
Moreover, Lauterbach et al. [74] concluded that food and chemical industries in Germany are the most appropriate for solar heat integration. They developed and analysed TRNSYS model simulation of German brewery for hot water supply by solar heating system [75]. Production systems can be improved for energy saving.
Soloha et al. [76] made an algorithm for possible use of solar thermal energy in district heating system in Latvia. They analysed a few possible scenarios. However, the highest problem is seasonal heat accumulation in tanks with hot water. The use of solar thermal energy in colder periods, especially in a geographic location as Latvia, can be very expensive.
The integration of solar heat in production processes can be done with different strategies. Many authors use solar heat integration as an additional subsystem in heat process integration with Pinch technology. Some of them designed independent solar heating systems connected to parts of manufacturing processes. It is known that solar heat stream depends on the intensity of incident solar radiation (insolation) and the time of insolation. This means solar heat stream can be taken as part of batch processes. There are two possible scenarios for integrating heat with other process streams. These potential scenarios are heat integration of batch processes with heat storage and without heat storage. However, the use of solar heat streams without heat storage is ridiculous. Always, there must be a found way to maximise the use of solar heat. On the other hand, heat storage is one of the main parts of solar heat integration.
Schmitt [77] made a classification of heat loads in the industry at the level of supply and processes. At the supply level, the heat loads are determined in steam and liquid heat transfer media. The process points of steam are steam generation, boiler feed water and make-up water. The heat transfer media includes parallel (direct or indirect) heat integration, increase of solar return flow and solar heating of heat storage or cascade. The heat loads at the level of the process are placed in conventional heating equipment. They are external heat exchanger (solar heating of product, Pinch methodology, intermediate hot water circuit, input streams, bath, machinery or tank), heating with internal heat exchanger, solar steam supply at vacuum or low pressure.
IEA reports about steps of solar heat integration in the industrial processes. These guidelines [23] are probably the most detailed description of solar heat process integration that can be found in literature. It suggested using Pinch technology or other advanced methodology for process integration. Appropriate solution can be found by systematic approach and optimisation. The major advantages are specification of bottlenecks, energy and mass efficiency solutions, planning of process modifications, optimally profitable solution for use of renewable energy supply in combination with heat recovery. IEA identified nine steps as a procedure for the implementation of solar heat integration. These steps are the following [23]:
Step 1: Pre-feasibility study – Basic data acquisition (use of different questionnaires to get basic information about possible solar heat integration);
Step 2: Pre-feasibility study – Preparation (collecting additional data about similar projects, clarifying the needs of company, etc.);
Step 3: Company visit;
Step 4: Analysis of status quo (engineering analysis and calculations: process flowsheets, energy balances, estimate energy consumption of single production sections or processes);
Step 5: Process optimisation and energy efficiency (investigation of energy saving potential of processes, heat recovery potential within utilities);
Step 6: Identification of integration points – applying the following criteria to the process: integration temperature levels, load profile, the amount of thermal energy consumed, effort for integration, sensitivity to changes, possible solar fractions. Furthermore, ranking of heat consumers based upon these criteria;
Step 7: Planning a Solar Heating for Industrial Processes (SHIP) system – an analysis of chosen suitable integration points with solar integration concepts (compared on economic and energetic basis). Basic design of solar heat systems with definition of collector field size, the storage volume and the type of solar thermal collectors;
Step 8: Comparative analysis decision – possibility for solar heat system (company decides on previous results about investment in solar heating system and the proposed solution);
Step 9: Detailed planning – starting a detailed planning based upon the company decision, repeating of some prior steps if that is necessary.
Steps 1-3 and 8-9 relate to any methodology used for integration of solar heating system with production processes. Steps 4-7 are specially related to methodologies, but the most to Pinch technology.
Solar heat integration is connected to the system through the integration points based on supply levels or process level [23]. Integration points in the real system represent a heat exchanger, a valve or a T-piece. They are determined from the suitability indicators of heat sinks. These indicators are the result of the pre-integration step. They are divided into three categories: heat demand, scheduling and technology determination. Heat demand is related to the process temperature (return temperature), temperature lift, annual heat demand, storage capacity, storage charging, operating time, mean load, and recirculation. The schedule can be created from coincidence daily demand, demand seasonality, demand uniformity, and rescheduling. The equipment indexes are related to supply quality of equipment and supply quality of the product.
According to IEA [23], there are a few very important parameters for solar heating systems, such as: integration flow temperature, integration return temperature and process flow temperature. Integration flow temperature is the value of temperature for solar heat supply stream at the integration point. This temperature can be defined for 3 different requirements as maximum flow temperature, set flow temperature and minimum flow temperature. The maximum flow temperature is specified value where the temperature of the solar heat supply stream can vary, depends on conditions. If the temperature of heating must be a constant value, that is set flow temperature. There is also minimum specified temperature at minimum flow. The integration return temperature is the value of temperature of solar heat stream when it leaves the integration point and goes back to the solar heat system. It could be constant or can vary from case to case. Process flow temperature can have maximal value. Its range of temperature tolerance is specified in the control system.
Baniassadi et al. [78] gave some other directions for solar heat integration. They separated variables as supply side variables and demand side variables. Supply side variables are collector parameters (area, array, type, flow rates) and parameters of heat storage (size, type, priority). The demand side is the production process (direct heating priority, ΔTmin for solar heat exchangers, flow rate of solar stream in the solar heat exchanger). The optimal flow rate of solar stream is determined to be the same as process flow. If that is not a case, it will lead to higher inlet or outlet temperatures of solar stream. This fact is based on temperature-enthalpy diagrams given by Baniassadi et al. [78]. An important thing is the priority between heating and heat storage. They suggested the storage of heat should be at minimal possible temperature to keep efficiency. They gave their opinion that direct heat exchange is more useful than heat storage for certain operating temperature intervals. That will cause better use of solar heat. In that case, the heat storage would have lower heat loss. Baniassadi et al. [79] suggested a method for heating-storage path that is based on temperatures of solar stream and process stream. The first point of solar stream is heat exchange with the process stream. The solar stream can be reused for another process stream and depends on its exit temperature. If the temperature is not satisfactory for heating any other process stream, it is going to the heat storage system. So, after the first priority point, the choice of the solar heat stream depends on the second priority. The second priority is returning back to the solar heating system or to another process stream for heat exchange. Based on that, these priority choices can be presented on Grand Composite Curve (GCC) as it is shown in Figure 2. They suggested the use of solar heat exchanger before or after already created Heat Exchanger Network (HEN) from process streams. Actually, that will reduce heat demands for utility. Frein et al. [49] made integration of solar heat plant in Tunisia textile industry with process integration of possible process energy streams. After that, they added solar heat plant into two different scenarios. There are cases with one and with two circuits for heat exchange. The case with one circuit consists of closed loop between the system of collectors and heat exchanger for heating water in the heat storage. Furthermore, hot water from heat storage tank is directly used for the production process. In the second case, there are two circuits. The first one is solar closed loop from the first case, and there is one additional closed loop. The second closed loop is using hot water from heat storage for indirect heating of process streams. Frein et al. [48] used the following 6 design steps:
Modelling of dying process and estimation of thermal consumption;
Monitoring of dying process consumption and validation of the model;
Construction of the load profile as input for plant simulation;
Modelling of solar thermal plant;
Sizing of the main components and choice of anti-stagnation control strategy;
Detailed design of solar thermal plant.
Similarly, Allouhi et al. [38] analysed water demand for processes that should be involved in the solar heat integration, set-point temperatures, returning temperatures, number of working hours, heat flow streams and number of working days per week. Set-point temperatures are temperatures that hot stream must have for heat supply. After the use of hot streams, they get returning temperature. The system contains solar modules connected with heat exchanger and heat storage tank. Heat storage tank is activated during the nights, and during cloudy days. There is also a back-up system. It is a heat supply system from fossil fuels. It is used when temperatures from the solar heating system have not satisfied the set-point values. The types of modules are flat plate and evacuated tube collectors with high performance. Water is the fluid for heat storage in the tank. The heat storage tank is connected with solar heating system via external heat exchanger. That subsystem supplies the heat storage with solar heat. There is also a back-up boiler connected in series with heat storage tank. Its duty is to keep constant set-point temperatures.
GCC for different solar stream priorities {demonstration of solar heat exchangers for heating-storage path (Baniassadi et al. [79])} (1. First priority, 2. Second priority, 3. Third priority, 4. Fourth priority)
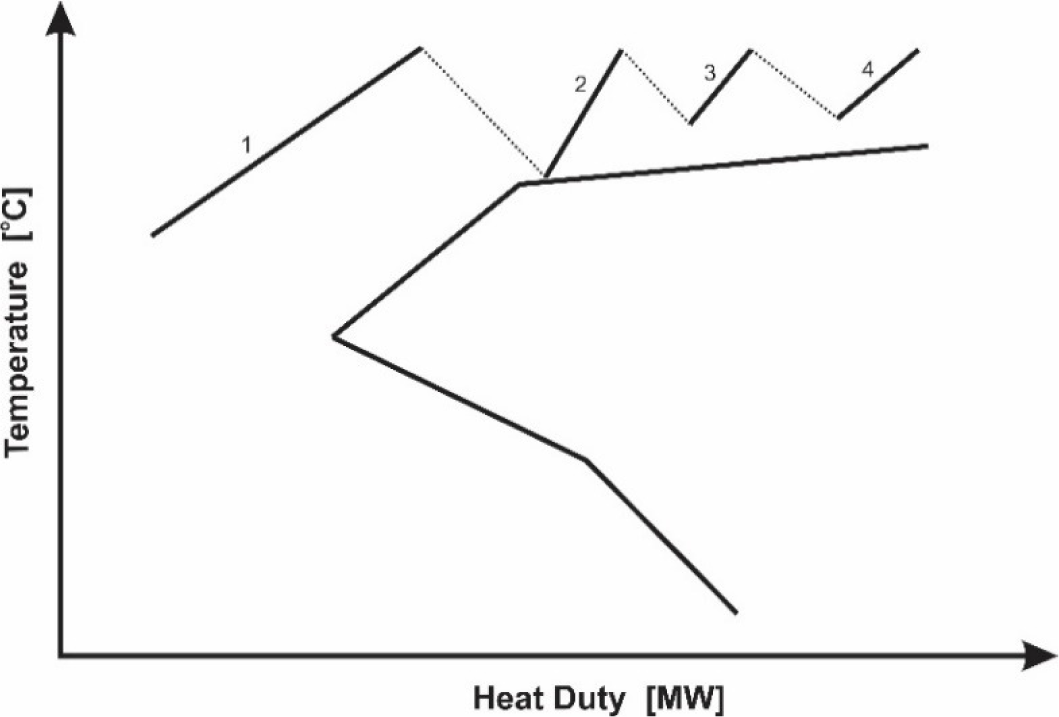
The basis of solutions for batch heat integration is Time Average Model (TAM), Time Sliced Model (TSM) and Problem Table Algorithm (PTA). These basic models are described by Linnhoff et al. [80] and later upgraded by Shenoy [81] and Kemp [82]. According to many authors, TSM and modified PTA are the closest ways to real solution of batch process integration. However, some analysis made on these models for solar heat integration showed different conclusions. Eiholzer et al. [59] used TSM and TAM for batch process integration with Pinch technology. They included solar heating system into the brewery production system. The methodology they used consisted of the following parts: pre-feasibility, feasibility study and decision-making. Feasibility study covers process optimisation with energy efficiency, and analysis of integration points (use of Pinch technology). In the case solved with TAM, solar thermal energy replaced 40% of hot utility. Eiholzer et al. [59] concluded that indirect heat recovery (TAM) by thermal energy storage can reduce the demand for the brewing process.
Varbanov et al. [83] enumerated a few cases of use of Pinch technology for improvement of energy efficiency. In general, Pinch analysis starts with data collection. That means determination of heating and cooling demands, main data of process streams (mass flow, heat capacity, supply and target temperatures). The next step is the determination of Pinch point as well as the optimal value of ΔTmin. Furthermore, everything is based on Pinch technology procedure, design of HENs (potential solutions) and selection of the best alternative. After completing this procedure, possible solar heat streams can be added. The solar heat stream can be added at two different levels, as utility stream (utility level) or process stream (process level). It can be integrated in different temperatures, based on the characteristics of the solar heating system. Involving of solar heat in process integration is made with design heat exchanger and heat storage system by use of algorithms for time-dependent processes (batch processes). Solar heat stream is included in Hot Composite Curve (HCC) and GCC, as it is shown on Figure 3. The place of solar stream segment in these curves depends on integration point (integration level). Moreover, there must be done an analysis for the remaining heat demand and redesign of HEN within solar process integration. All these procedures are shown in the scheme (Figure 4).
Solar heating stream segments in: HCC (a) and GCC (b) based on integration level (different temperature levels) [23]
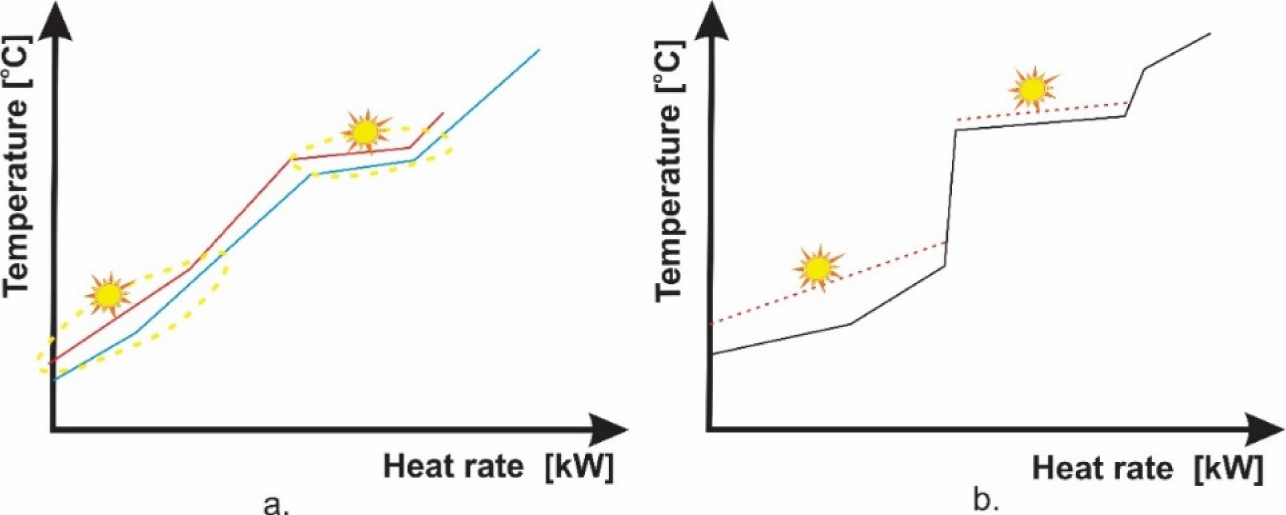
Simplified workload for Pinch technology in solar heat integration [23]
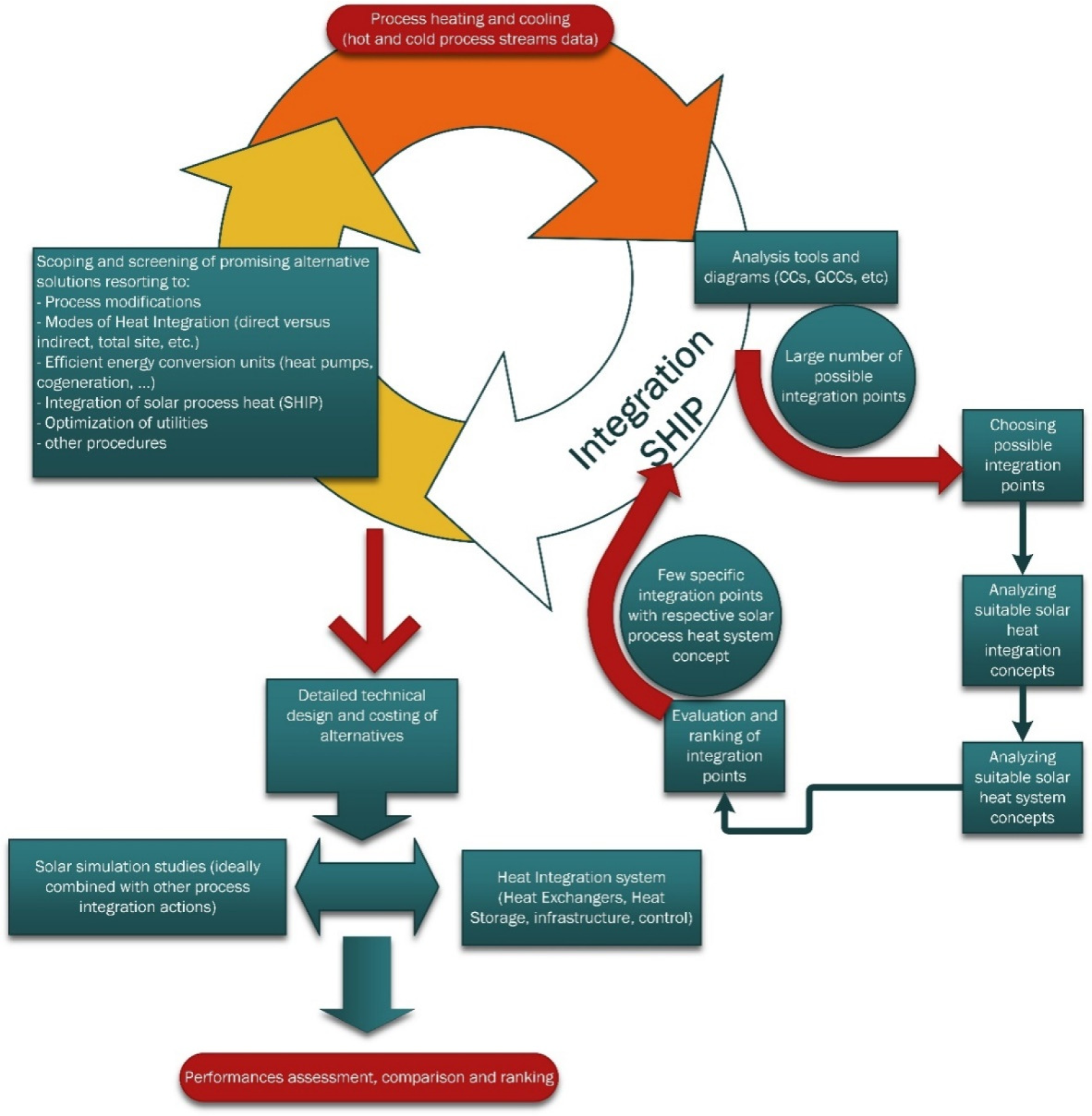
The approach given by the IEA can be upgraded with some additional parts. Varbanov [84], as well as Varbanov and Klemeš [85] developed an extended total site methodology for integration in cases of varying energy supply and demand. This methodology is related to different production processes. It is addressed to renewable energy sources. They exist periodically. Varbanov determined time slices from TSM for batch processes. His extended methodology covers analysis of temporal variations in the heating and supply demands, obtains the total site utility targets with total site heat cascade, total site profiles and site composite curves, energy storage capacity targeting. Liew et al. [86] updated this methodology for variable energy demand/supply. They suggested algorithmic targeting of time slices. The first step is time slice identification. After identification, multiple utility targets for all time slices must be determined. The next step is the construction of Total Site Heat Storage Cascade (TSHSC). TSHSC is important for the calculation of the total amount of heat that can be recovered within time slices. That heat would be stored in heat storage with duties for heat supply of different time slices. Varbanov and Klemeš [85] used this methodology in Locally Integrated Energy Sector (LIES). They integrated two industrial plants, a hotel and local district heating. Nemet et al. [87] introduced two novel curves for the trade-off between captured solar heat and process demand. These new curves are Captured Solar Energy Curve (CSEC) and Minimal Capture Temperature Curve (MCTC). The first one represents available solar heat. MCTC determines the minimum temperature that makes heat transfer feasible. The crossing point between these two curves is minimal temperature that is still sufficiently high to be useable in the production process. Nemet et al. [87] targeted the maximal solar heat based on a simple system of solar collector and heat storage. There, the heat storage can be based on thermal heat storage or chemical energy storage. So, they defined direct and indirect heat transfer from solar collectors. The two new curves, CSEC and MCTC are involved in process heat integration tools. The CSEC can be constructed as a result of interpolation between points that present the maximum available solar thermal energy at certain temperatures (Figure 5). Nemet et al. [87] constructed MCTC curve by using algorithm shown on Figure 6.
Possible streams for solar thermal energy and constructing of CSEC [87]: possible stream fora transfer from solar capture system to storage (a) and set possible streams for solar thermal energy as hot utility (b)
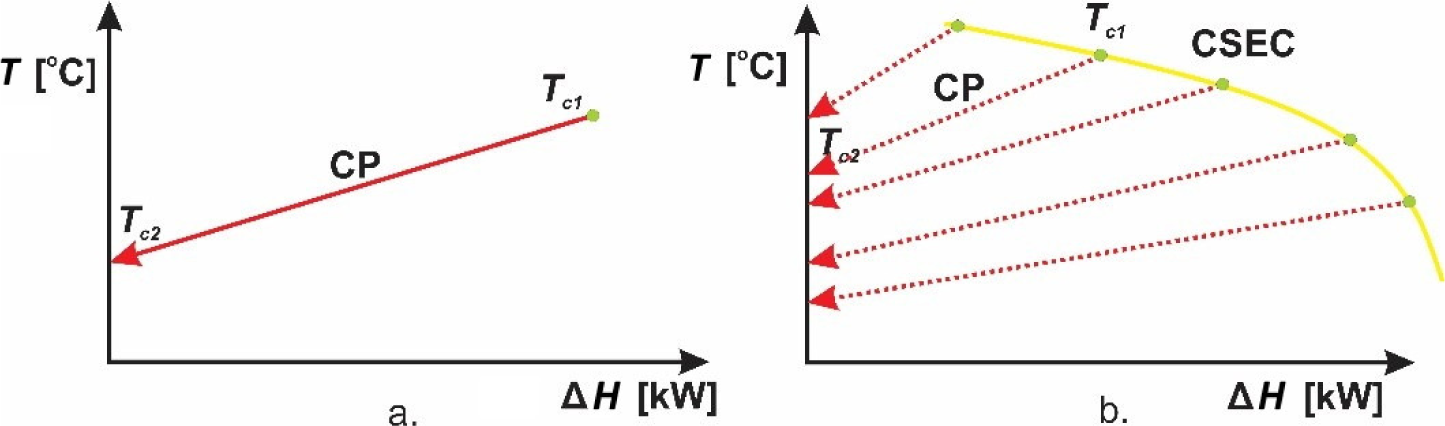
Algorithm for construction of MCTC given by Nemet et al. [87]

The position of the solar heat stream is very important for integration. Atkins et al. [88] analysed two different cases for solar heat integration with Pinch technology. They considered either constant flow or constant outlet temperature for collector systems. The results are examined for solar heat integrated above and below the Pinch. Only the solar heating system integrated above Pinch has modest heat savings in New Zealand milk powder production plant. Otherwise, integration below Pinch is impossible. That is the incorrect way. In case when mass integration is added to the heat integration process, savings are greater.
Furthermore, Walmsley et al. [89] used two different approaches to solar heat storage for their integration of dairy production processes. They made calculations with constant temperature of heat storage, as well as variable temperature of heat storage. In the case of constant temperature of solar heat stream, they used average values for streams. Walmsley et al. [89] created new modified method for cases with variable temperature of heat storage. A case with changeable heat storage temperature is examined on a daily based time average composite curve. These calculations are related to systems with Heat Recovery Loop (HRL). Their method is described with the following steps:
Data extraction (heat recovery, additional ΔT, data from heat streams);
Construction of composite curves with determination of cold and hot stream outlet temperature;
Calculation of limited values for heat capacity flows, ΔTmin, and selection of limited temperatures for hot and cold streams;
Scaling of limited heat capacity flows;
Determination of heat exchange area.
These steps are used in targeting and creating of transient HRL model with Variable Temperature Storage (VTS).
Schmitt [77] found a few conditions that are very important for solar heat integration. They are distinction between supply and process level, heat transfer medium at supply level, category of heat consumer at process level, and the conventional way of heating at process level. These are the basis for the choice of solar heat integration concept. In case when steam is the purpose of solar heat use, the following concepts exist: solar steam generation (parallel integration), heating of boiler feed water (serial integration), heating of make-up water (serial integration). When some liquid media is used for heat transfer, parallel integration is chosen. That will increase the return flow and storage or cascade heating. If solar heat integration is on a process level, some of the following categories could be selected: (pre)heating of fluid streams, heating and maintaining temperature of baths, machinery and tanks, and thermal separation processes based on boiling point. The solar heat integration concepts for these categories at process level are heating of the process fluids, heating of the intermediate hot-water circuit, heating of bath, machinery or tank, heating of input streams, and vacuum steam and low-pressure steam.
The term “polygeneration” means production of energy from various outputs and by-products made by one or more energy inputs. This can cause high degree of process integration. Picón-Núñez and Medina-Flores [90] studied polygeneration integrated into production processes. The higher degree of process integration can be found in single sources of energy generation, such as gasification of coal or heavy oil, biogas production from biomass, as well as bioethanol production process. They determined the rise of energy efficiency in utilities made with polygeneration. If utilities are cogenerated in three systems as one, the benefit is higher. In case when utilities are made into stand-alone systems, efficiency is lower. Berntsson et al. [91] included Pinch analysis in heat and power utility systems. They proposed combination of different heat engines to be integrated into the production processes. There is the possibility for combined heat and power use in steam turbines, gas turbines, combined gas turbine and steam network, and ORC.
Gencer and Agrawal [92] designed so-called synthesise Solar Electricity, Water, Food and Chemical (SEWFAC) processes. This system practically contains a cogeneration concept. The SEWFAC is based on Food, Energy, Water (FEW) nexus for sustainability (Figure 7). There is a big difference between SEWFAC approach and conventional ways. SEWFAC takes all energy and water sources together for integration with the production process. The conventional processes integrate process separately by the resource type. The SEWFAC methodology created by Gencer and Agrawal [92] analyse the production system for exergy lost and process design with optimisation (Pinch points determination). After that, each process should be examined for potential in multi-purpose integration. The main optimisation is made with exergy analysis. Gencer and Agrawal [92] implemented their approach in hydrogen production system with solar energy. They made process simulation in MATLAB and Aspen Plus with interaction between them. Aspen Plus is used for basic model and iterative model. They used MATLAB for simulation of solar thermal production of hydrogen, heat integration of the system and efficiency calculation. MATLAB application is initiated and terminated with genetic algorithm as optimisation method for determination of maximum efficiency. The cogeneration system includes High-Pressure Turbine (HPT) and Medium Pressure Turbine (MPT) for electricity production.
FEW nexus for sustainability
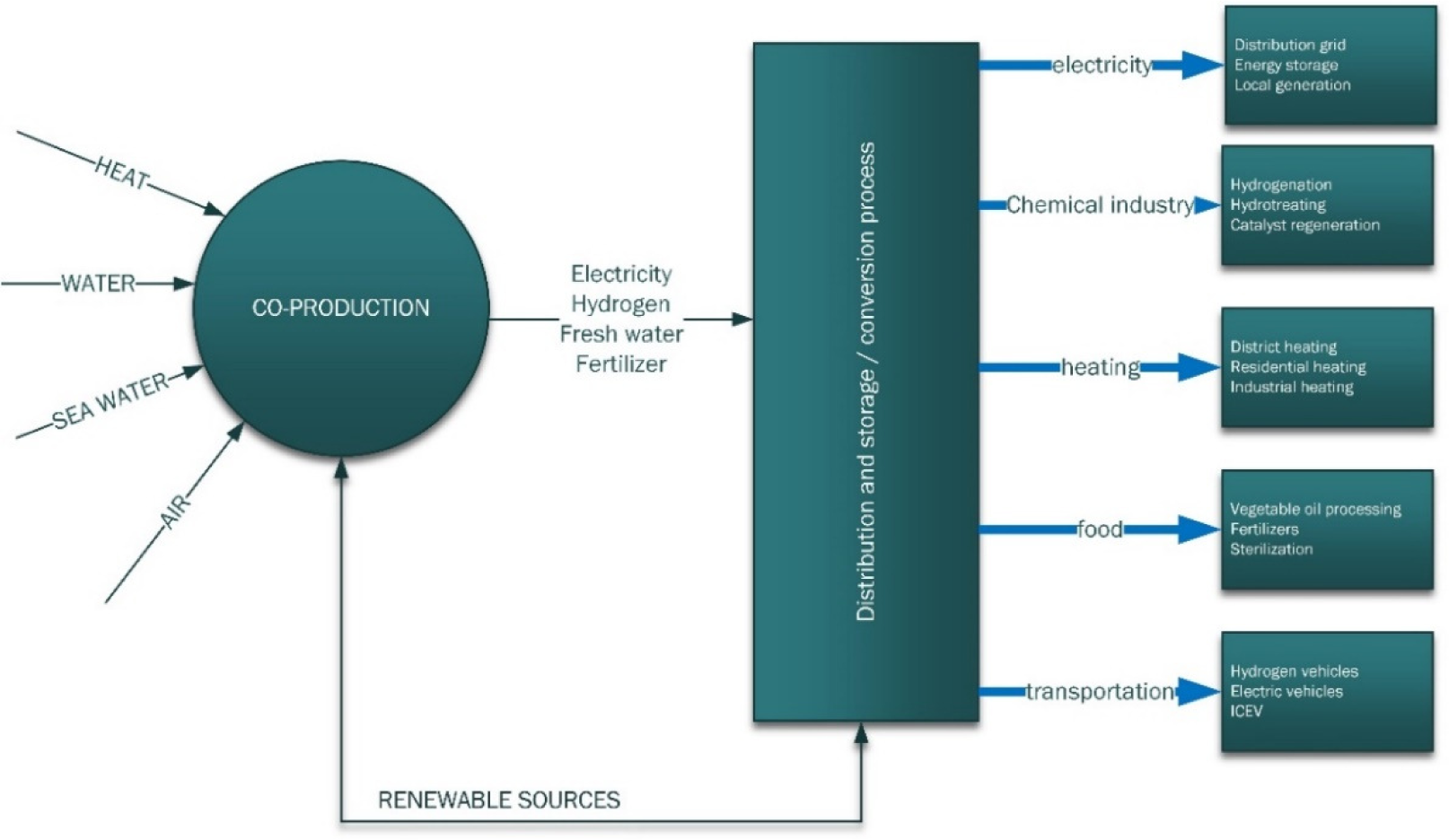
Arabkoohsar and Andresen [93] designed a novel system for trigeneration – heat, cooling and power. They used subcooled compressed air energy storage in combination with a solar-powered absorption chiller. Furthermore, Chen et al. [94] analysed solar assisted combined power and cooling system. In that process, solar heating system and internal combustion engine are heat sources. All that heat is transferred to the heat recovery vapour generator. Vapour is going through turbine that is connected with two refrigeration subsystems.
Sadi and Arabkoohsar [95] proposed combination of solar heating system and incineration plant for getting free heat energy. That heat is supplied to Rankine cycle (three turbines in series – high pressure, intermediate pressure and low-pressure turbines). Turbines are connected with electricity generator. The heat generated from the sun and incineration is transformed into electricity.
Moreover, polygeneration is possible in farms. Rikkonen et al. [96] used Delphy method for research the potential of renewable energy sources that can be used on farms in Finland. They determined following parts of the method: commenting on pre-prepared alternative agricultural climate and energy scenario narratives, evaluating agricultural climate change mitigation measures, anticipating the energy change scenarios of self-sufficient farm-based renewable energy production, and anticipating the business opportunities as well as the obstacles and solutions in the growth of farm-based energy production in Finland. But this is more related to agriculture than industry.
Some authors use different methods for solar heating integration than all mentioned before. Müller et al. [41] developed methodology for solar heat integration in German industry. That is based on experience from German brewery and dairy plants. It consists of 5 main steps: analysis and evaluation of statistical data, selection of typical companies, roof analysis with evaluation matrix, evaluation and generalisation of results, definition of subsector potential for solar thermal energy use. This method is based on use of the so-called “evaluation matrix”. Müller et al. [41] analysed the type of roof, the side of collector position (north-south), available roof area for collector system, and shading. According to all these data, the maximum possible collector area can be calculated. The active collector area is the parameter that is used for predicting the possibilities in solar thermal accumulation.
Wallerand et al. [39] have used a different approach to this problem. They made solar heat process integration by using multi-period Mixed Integer Linear Programming (MILP). Their study is based on the following steps: data collection (utility and process data, energy and mass balances, thermodynamic models, meteorological data), system resolution (targeting different utility designs by solving MILP based on minimising total annual cost), performance calculation (some non-linear related parameters as well as the Carnot efficiency index recalculation – thermo-environomic calculations). They found the final solution by doing this procedure in many iterations.
Software based on optimisation methods is also useful. Allouhi et al. [38] analysed their system with TRANSOL software. They found that the final solution gives good performance and profitability.
All approaches in solar heat integration are summarised in Figure 8. All mentioned major methodologies were placed there. The direction for creating the best connection between solar heating systems and production processes are shown. As it can be seen, the most detailed analysis can be done with Pinch technology. The Pinch technology can interact with other mentioned methods.
Here, the general approach can be given for steps in the beginning of the integration process (pre-feasibility stadium) and steps at the end of the integration (post-feasibility stadium). Every methodology is starting with data acquisition for plant that should be integrated with solar heating system. Requirements of company and possible solutions for the company request should be analysed, as well as needs for overview of terrain (visit of company). All steps in the beginning (steps 1-3) are pre-feasibility stage of the project for solar heat integration.
The real work on project starts with analysis of “status quo” and data analysis. In general, extracted data can suggest the main direction of choosing the right method.
If there is need for solar heat integration with process streams, Pinch technology or exergy analysis should be selected. The exergy analysis finds the points of exergy loss. In that case, Pinch point should be found and design of solution based on minimum exergy loss. That process level interacts with integration level (identification of potential integrations, synthesise integration and intensification of the production process). Their interaction evaluates suitable technologies to apply. Integration with Pinch technology is more complex. Many authors upgrade that methodology. Mainly, solar heat integration is taken as batch process, but in case of constant use of heat storage it can be used for design of continuous processes or solutions that use TAM. Time sliced model is the most accurate for batch processes. All steps in Pinch technology are shown on Figure 8 and they are the basic steps combined with some additional analysis like total site utility target with total site heat cascade, site composite curves, heat storage design and different approaches in HEN design as a final solution.
General view of mostly used methodologies for solar thermal integration
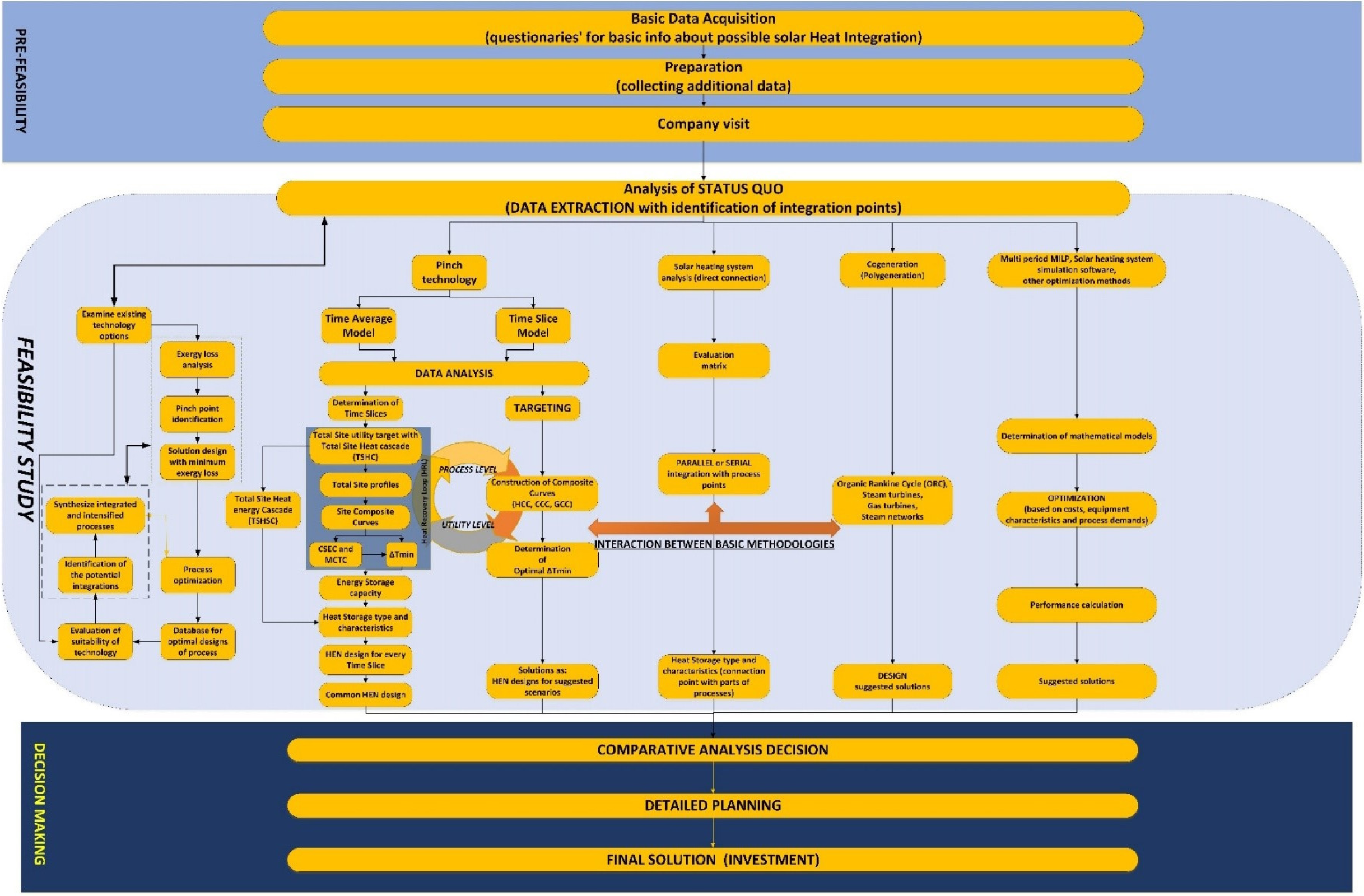
If the purpose of solar heating is the generation of hot water or steam in production, there should be used direct connection of solar heat system and streams involved in utility production. It can be done with the evaluation matrix and in two ways of connection (parallel and serial connection). Polygeneration can suggest using of combination of methodologies. Practically, already integrated production system with solar heating system can be combined with different heat pumps in design of polygeneration system. It is special integration of ORC types, steam turbines, gas turbines, absorption chillers, steam networks, etc.
The optimisation methods can be used in production systems, but they will not always give good results. There are many cases where process systems are optimised with combination of these methods and Pinch technology or direct connection of solar heating system.
Generally speaking, all the methods mentioned in the text reduce production costs by integrating solar thermal systems. The authors of the cited papers mostly focus on techniques for processes optimisation and minimising the use of thermal energy. Few of them provide detailed information on the feasibility of projects with integrated solar thermal systems. A very important economic parameter is the payback period. Logically, in case of replacement of greater amount of heat generated from fossil fuels will have more positive effect to the investment. But a very important factor is capital cost. Capital costs comprise the basic investment in machinery and equipment. They depend on the type of solar collector or concentrator, the use of heat storage, and possible use of additional types of equipment that can be very expensive. Also, very important fact in design and use of solar heating systems are solar insolation data for certain geolocations. The investments for solar heating systems in locations of northern Europe are not so profitable because of low insolation and need of a very large area of solar collectors or concentrators. The plant life of solar thermal systems is estimated between 20-30 years and depends on equipment used in it.
The simplest methods that involve just connecting a solar thermal system to certain points in production have a very low investment cost, especially if there is a need for hot processing water. Steam generation in solar heating systems or fluids with high temperatures increases the cost of investment. Such systems can be attractive for production on daylight.
If the production system is optimised with Pinch technology or some other optimisation method for waste heat reuse, the integration of solar thermal systems is much more attractive with higher profits and less investment. Cases that include heat storage increases the cost. That cost depends on heat storage volume, the heat storage material and the process used for heat storage (latent heat, transformation to electricity and store in batteries, chemical reaction or production of environment-friendly fuel like hydrogen) [97]. Allouhi et al. [38] made economic analysis of their optimal design for integrated solar thermal system in food factory in Casablanca. The payback period is determined as 12.27 years, but this period is subject to alteration. Furthermore, Eiholzer et al. [59] used Pinch technology for solar heat integration in a brewery. There, hot water is needed below 100 °C. The economic analysis shows payback period of 6.4 years. Quijera and Labidi [36] also gives optimistic predictions for profitability in heat integration of dairy industry with use Pinch and exergy analysis.
In case of polygeneration, the situation is much different. The opportunities of heat reuse and its transformation into electricity or other type of energy is higher. But the cost of equipment is much higher than in applying of other approaches. Including ORC or similar thermodynamic cycles can increase the capital cost to get a case that is not profitable at all. The prices of ORC or other types of equipment for polygeneration are very high. One of the largest investments in ORC in cogeneration with wood waste is made in Lienz, Austria. The report for that plant [98] shows low profitability without help by the Austrian institutions and the law.
Timilsina et al. [99] compared solar energy technologies and conventional technology that use natural gas, coal and oil. They have shown that the solar energy has a high potential, despite the fact that the capital costs of conventional use of coal, natural gas and oil are much lower. Based on the environmental impact and free solar energy, the new renewable energy technologies are very attractive.
This paper summarises different approaches in solving the problem of solar thermal energy integration in production systems. Researchers use different methodologies. Many authors based their projects or feasibility studies on Pinch technology. There are different modifications that are related to specific production processes. General strategies for solar heat integration are given only by the IEA. However, they leave opportunities for using Pinch technology or another optimisation method.
Results of projects published in articles, which are used for this study have a variety of positive and negative experiences. Many projects are done in simplest way with direct use of generated hot water from the solar heating system. Anyway, the use of solar heating systems replaces parts of heat demand in production processes. That reduces the use of fossil fuels and decreases the pollution gas emissions. The main problem in solar heat integration in production processes has location, fossil fuel prices and problems in heat storage for a longer period. Every location has a specific annual insolation. Northern countries have lower possibilities in solar heat use. Sometimes it could be more expensive than conventional systems. The price of natural gas can be an economically better solution than investing in very large equipment for solar heat collection. Finally, heat storage systems are out of ideal storing of thermal energy in a longer period.
The imperfection of solar heating system is not the reason for rejecting projects. Countries that have locations with relatively high insolation can make very profitable integration into industrial production systems. Many projects from Germany, Austria, Switzerland, Mediterranean countries and Middle East approved that.
At the end, there are many gaps present in solar heating integration. The most important gap is solving of heat storage for longer periods. That will help in using solar heat at night and in case of bad weather. Also, the possibility for integration of different heat sources and sinks with solar heating systems for different purposes have many problems. The temperature range is a problem in use of heat engines and turbines. Also, integration with industrial batch processes has specific needs. The solar heat stream is periodic. The daily insolation on sunny days is different (winter/summer time). Heat streams from solar heating systems have characteristics of changeable streams involved in batch processes.
Cp | heat capacity | [kJ/kg K] |
np | Nanoparticle |
bf | bulk fluid |
nf | Nanofluid |
ρ | density | [kg/m3] |
φ | mass ratio | [-] |
CPVT | Combined Photovoltaic – Thermal collectors |
CSEC | Captured Solar Energy Curve |
CSP | Concentrated Solar Power |
DSG | Direct Steam Generation |
GCC | Grand Composite Curve |
HCC | Hot Composite Curve |
HEN | Heat Exchanger Network |
HRL | Heat Recovery Loop |
IEA | International Energy Agency |
LCC | Life Cycle Costs |
LCT | Life Cycle Thinking |
LIES | Locally Integrated Energy Sector |
MCTC | Minimal Capture Temperature Curve |
MILP | Multiple Integer Linear Programming |
ORC | Organic Rankine Cycle |
PTA | Problem Table Algorithm |
RO | Reverse Osmosis |
SHIP | Solar Heat Integration in Production |
TAM | Time Average Model |
TES | Thermal Energy Storage |
TPES | Total Primary Energy Supply |
TSHI | Total Site Heat Integration |
TSHSC | Total Site Heat Storage Cascade |
TSM | Time Sliced Model |
VTS | Variable Temperature Storage |
BP Statistical Review of World Energy 2017 , 2016
, Key World Energy Statistics 2017 , 2017
, Task 49: Solar Heat for Industry: Great Potential Ahead , 2017
, , Solar Energy Engineering: Processes and Systems , 2014
, A Review on Development of Solar Drying Applications ,Renew. Sustain. Energy Rev. , Vol. 31 ,pp 133-148 , 2014, https://doi.org/https://doi.org/10.1016/j.rser.2013.11.052
, Solar Process Heat in Industrial Systems – A Global Review ,Renew. Sustain. Energy Rev. , Vol. 82 (Part 3),pp 2270-2286 , 2018, https://doi.org/https://doi.org/10.1016/j.rser.2017.08.065
, Solar Industrial Process Heating Systems in Operation – Current SHIP Plants and Future Prospects in Australia ,Renew. Sustain. Energy Rev. , Vol. 91 ,pp 409-419 , 2018, https://doi.org/https://doi.org/10.1016/j.rser.2018.03.105
, Status and Prospect of Solar Heat for Industrial Processes in China ,Renew. Sustain. Energy Rev. , Vol. 90 ,pp 475-489 , 2018, https://doi.org/https://doi.org/10.1016/j.rser.2018.03.077
, Tri and Polygeneration Systems ‒ A Review ,Renew. Sustain. Energy Rev. , Vol. 60 ,pp 1032-1051 , 2016, https://doi.org/https://doi.org/10.1016/j.rser.2016.01.127
, Renewable Energy Technologies Adopted by the UAE: Prospects and Challenges – A Comprehensive Overview ,Renew. Sustain. Energy Rev. , Vol. 55 ,pp 1181-1194 , 2016, https://doi.org/https://doi.org/10.1016/j.rser.2015.05.087
, A Review of Solar Thermochemical Processes ,Renew. Sustain. Energy Rev. , Vol. 54 ,pp 497-532 , 2016, https://doi.org/https://doi.org/10.1016/j.rser.2015.10.026
, Review and Multi-Criteria Assessment of Solar Energy Projects in Chile ,Renew. Sustain. Energy Rev. , Vol. 59 ,pp 583-596 , 2016, https://doi.org/https://doi.org/10.1016/j.rser.2015.12.149
, Categorization and Analysis of Heat Sources for Organic Rankine Cycle Systems ,Renew. Sustain. Energy Rev. , Vol. 64 ,pp 790-805 , 2016, https://doi.org/https://doi.org/10.1016/j.rser.2016.06.076
, New Directions in the Implementation of Pinch Methodology (PM) ,Renew. Sustain. Energy Rev. , Vol. 98 ,pp 439-468 , 2018, https://doi.org/https://doi.org/10.1016/j.rser.2018.09.030
, Life Cycle Thinking (LCT) Applied to Residential Heat Pump Systems: A Critical Review ,Energy and Buildings , Vol. 185 ,pp 210-223 , 2019, https://doi.org/https://doi.org/10.1016/j.enbuild.2018.12.035
, Economical Assessment and Applications of Photovoltaic/Thermal Hybrid Solar Technology: A Review ,Solar Energy , Vol. 153 ,pp 540-561 , 2017, https://doi.org/https://doi.org/10.1016/j.solener.2017.05.081
, Solar Energy For Future World: ‒ A Review ,Renew. Sustain. Energy Rev. , Vol. 62 ,pp 1092-1105 , 2016, https://doi.org/https://doi.org/10.1016/j.rser.2016.05.022
, Greening Ammonia Toward the Solar Ammonia Refinery ,Joule , Vol. 2 (6),pp 1055-1074 , 2018, https://doi.org/https://doi.org/10.1016/j.joule.2018.04.017
, Potential of Solar Energy in Developing Countries for Reducing Energy-Related Emissions ,Renew. Sustain. Energy Rev. , Vol. 90 ,pp 275-291 , 2018, https://doi.org/https://doi.org/10.1016/j.rser.2018.03.065
, Solar Industrial Process Heat ,Ansole Webinar , 2011
, , Solar Thermal and Biomass Energy , 2010
, Solar Energy for Process Heating: A Case Study of Select Indian Industries ,J. Clean. Prod. , Vol. 151 ,pp 439-451 , 2017, https://doi.org/https://doi.org/10.1016/j.jclepro.2017.02.190
, Solar Process Heat for Production and Advanced Applications (Integration Guideline), IEA SHC Task 49, SolarPACES Annex IV , 2014
, , Control of Solar Energy Systems , 2012
, Design of Heat Storage Units for Use in Repeatable Time Slices ,Appl. Therm. Eng. , Vol. 112 ,pp 1590-1600 , 2017, https://doi.org/https://doi.org/10.1016/j.applthermaleng.2016.10.086
, The Release Properties of Nitrogen Oxides of Solar Salt Used for Thermal Energy Storage in Different Environment ,Energy Procedia , Vol. 105 ,pp 4420-4427 , 2017, https://doi.org/https://doi.org/10.1016/j.egypro.2017.03.937
, Applications of Nanofluids in Solar Energy: A Review of Recent Advances ,Renew. Sustain. Energy Rev. , Vol. 82 (Part 3),pp 3483-3502 , 2018, https://doi.org/https://doi.org/10.1016/j.rser.2017.10.108
, A Review of Entropy Generation in Nanofluid Flow ,Int. J. Heat Mass Transf. , Vol. 65 ,pp 514-532 , 2013, https://doi.org/https://doi.org/10.1016/j.ijheatmasstransfer.2013.06.010
, Nanofluid-Based Direct Absorption Solar Collector ,J. Renew. Sustain. Energy , Vol. 2 (3), 2010, https://doi.org/https://doi.org/10.1063/1.3429737
, An Experimental Investigation on the Effect of Al2O3-H2O Nanofluid on the Efficiency of Flat-Plate Solar Collectors ,Renew. Energy , Vol. 39 (1),pp 293-298 , 2012, https://doi.org/https://doi.org/10.1016/j.renene.2011.08.056
, Improvement in the Performance of Solar Collectors With Nanofluids ‒ A State-of-the-Art Review ,Nano-Structures & Nano-Objects , Vol. 18 , 2019, https://doi.org/https://doi.org/10.1016/j.nanoso.2019.100276
, Experimental Investigation on the Thermal Efficiency and Performance Characteristics of a Flat Plate Solar Collector Using SiO2/EG-Water Nanofluids ,Int. Commun. Heat Mass Transf. , Vol. 65 ,pp 71-75 , 2015, https://doi.org/https://doi.org/10.1016/j.icheatmasstransfer.2015.02.011
, Experimental Investigation on the Efficiency of Flat-Plate Solar Collectors With Nanofluids ,Appl. Therm. Eng. , Vol. 88 ,pp 165-171 , 2015, https://doi.org/https://doi.org/10.1016/j.applthermaleng.2014.09.053
, Energy, Economic and Environmental Analysis of Metal Oxides Nanofluid for Flat-Plate Solar Collector ,Energy Convers. Manag. , Vol. 76 ,pp 162-168 , 2013, https://doi.org/https://doi.org/10.1016/j.enconman.2013.07.038
, Integration of a Solar Thermal System in Canned Fish Factory ,Appl. Therm. Eng. , Vol. 70 (2),pp 1062-1072 , 2014, https://doi.org/https://doi.org/10.1016/j.applthermaleng.2014.04.012
, Pinch and Exergy Based Thermosolar Integration in a Dairy Process ,Appl. Therm. Eng. , Vol. 50 (1),pp 464-474 , 2013, https://doi.org/https://doi.org/10.1016/j.applthermaleng.2012.06.044
, Process and Economic Optimisation of a Milk Processing Plant With Solar Thermal Energy ,Comput. Aided Chem. Eng. , Vol. 38 ,pp 1347-1352 , 2016, https://doi.org/https://doi.org/10.1016/B978-0-444-63428-3.50229-0
, Design Optimization of a Multi-Temperature Solar Thermal Heating System for an Industrial Process ,Appl. Energy , Vol. 206 ,pp 382-392 , 2017, https://doi.org/https://doi.org/10.1016/j.apenergy.2017.08.196
, Optimal Design of Solar-Assisted Industrial Processes Considering Heat Pumping: Case Study of a Dairy ,Renew. Energy , Vol. 128 (Part B),pp 565-585 , 2018, https://doi.org/https://doi.org/10.1016/j.renene.2017.07.027
, Manufacture of Malt and Beer with Low Temperature Solar Process Heat ,Energy Procedia , Vol. 48 ,pp 1188-1193 , 2014, https://doi.org/https://doi.org/10.1016/j.egypro.2014.02.134
, Development of an Evaluation Methodology for the Potential of Solar-thermal Energy Use in the Food Industry ,Energy Procedia , Vol. 48 ,pp 1194-1201 , 2014, https://doi.org/https://doi.org/10.1016/j.egypro.2014.02.135
, Development of Hybrid Solar Distillation System for Essential Oil Extraction ,Renew. Energy , Vol. 113 ,pp 22-29 , 2017, https://doi.org/https://doi.org/10.1016/j.renene.2017.05.027
, Industrial Energy Usage in Australia and the Potential for Implementation of Solar Thermal Heat and Power ,Energy , Vol. 43 (1),pp 261-272 , 2012, https://doi.org/https://doi.org/10.1016/j.energy.2012.04.031
, Solar Industrial Process Heating in Australia – Past and Current Status ,Renew. Energy , Vol. 36 (1),pp 216-221 , 2011, https://doi.org/https://doi.org/10.1016/j.renene.2010.06.023
, Monitoring Results of a Solar Process Heat System Installed at a Textile Company in Southern Germany ,Energy Procedia , Vol. 70 ,pp 615-620 , 2015, https://doi.org/https://doi.org/10.1016/j.egypro.2015.02.168
, Investigation on the Feasibility of Integration of High Temperature Solar Energy in a Textile Factory ,Renew. Energy , Vol. 36 (12),pp 3517-3529 , 2011, https://doi.org/https://doi.org/10.1016/j.renene.2011.06.001
, Solar Thermal Plants for Industrial Process Heat in Tunisia: Economic Feasibility Analysis and Ideas for a New Policy ,Energy Procedia , Vol. 30 ,pp 1390-1400 , 2012, https://doi.org/https://doi.org/10.1016/j.egypro.2012.11.153
, Solar Thermal Plant Integration Into an Industrial Process ,Energy Procedia , Vol. 48 ,pp 1152-1163 , 2014, https://doi.org/https://doi.org/10.1016/j.egypro.2014.02.130
, Tubular Solar-Energy Collector Integration: Performance Enhancement of Classical Distillation Unit ,Energy , Vol. 141 ,pp 818-838 , 2017, https://doi.org/https://doi.org/10.1016/j.energy.2017.09.110
, Industrial Solar Process Heat Yield and Feasibility ,Proceedings of the 3rd Southern African Solar Energy Conference (SASEC2015) ,pp 112-117 , 2015
, Thermal Energy Storage Evaluation in Direct Steam Generation Solar Plants ,Sol. Energy , Vol. 159 ,pp 501-509 , 2018, https://doi.org/https://doi.org/10.1016/j.solener.2017.11.006
, Monitoring of a MW Class Solar Field Set up in a Brick Manufacturing Process ,Energy Procedia , Vol. 48 ,pp 1217-1225 , 2014, https://doi.org/https://doi.org/10.1016/j.egypro.2014.02.138
, Concrete Thermal Energy Storage for Linear Fresnel Collectors: Exploiting the South Mediterranean’s Solar Potential for Agri-Food Processes ,Energy Convers. Manag. , Vol. 166 ,pp 719-734 , 2018, https://doi.org/https://doi.org/10.1016/j.enconman.2018.04.075
, Solar Energy and Cork: A Binomial of the Future? ,Sci. Technol. Mater. , Vol. 30 (Suppl. 1),pp 80-86 , 2018, https://doi.org/https://doi.org/10.1016/j.stmat.2018.11.004
, Thermodynamic Analysis of a Novel Solar-Hybrid System for Low-Rank Coal Upgrading and Power Generation ,Energy , Vol. 141 ,pp 1737-1749 , 2017, https://doi.org/https://doi.org/10.1016/j.energy.2017.11.046
, On the Outlook for Solar Thermal Hydrogen Production in South Africa ,Int. J. Hydrogen Energy , Vol. 44 (2),pp 629-640 , 2019, https://doi.org/https://doi.org/10.1016/j.ijhydene.2018.11.069
, Total Site Heat Integration Planning and Design for Industrial, Urban and Renewable Systems ,Renew. Sustain. Energy Rev. , Vol. 68 (Part 2),pp 964-985 , 2017, https://doi.org/https://doi.org/10.1016/j.rser.2016.05.086
, A Methodology to Integrate Solar Thermal Energy in District Heating Networks Confronted With a Swedish Real Case Study ,Energy Procedia , Vol. 122 ,pp 865-870 , 2017, https://doi.org/https://doi.org/10.1016/j.egypro.2017.07.451
, Integration of a Solar Thermal System in a Medium-Sized Brewery Using Pinch Analysis: Methodology and Case Study ,Appl. Therm. Eng. , Vol. 113 ,pp 1558-1568 , 2017, https://doi.org/https://doi.org/10.1016/j.applthermaleng.2016.09.124
, Potential for Energy Efficiency Measures and Integration of Renewable Energy in the European Food and Beverage Industry Based on the Results of Implemented Projects ,Energy Procedia , Vol. 123 ,pp 148-155 , 2017, https://doi.org/https://doi.org/10.1016/j.egypro.2017.07.243
, Development of a Novel Solar-Based Integrated System for Desalination With Heat Recovery ,Appl. Therm. Eng. , Vol. 129 ,pp 1618-1633 , 2018, https://doi.org/https://doi.org/10.1016/j.applthermaleng.2017.09.028
, Optimization of Solar Integration in Biomass Fuelled Steam Plants ,Energy Procedia , Vol. 81 ,pp 390-398 , 2015, https://doi.org/https://doi.org/10.1016/j.egypro.2015.12.108
, Optimal Design and Integration of Solar Thermal Collection, Storage, and Dispatch With Process Cogeneration Systems ,Chem. Eng. Sci. , Vol. 136 ,pp 158-167 , 2015, https://doi.org/https://doi.org/10.1016/j.ces.2015.04.043
, Integration of Waste Heat and Renewable Sources of Energy in the Crude Oil Atmospheric Distillatio Plant ,Ph.D. Thesis , 2017
, An Assessment of Solar-Powered Organic Rankine Cycle Systems for Combined Heating and Power in UK Domestic Applications ,Appl. Energy , Vol. 138 ,pp 605-620 , 2015, https://doi.org/https://doi.org/10.1016/j.apenergy.2014.10.035
, Heat Recovery of Nano-Fluid Based Concentrating Photovoltaic Thermal (CPV/T) Collector With Organic Rankine Cycle ,Energy Convers. Manag. , Vol. 179 ,pp 373-396 , 2019, https://doi.org/https://doi.org/10.1016/j.enconman.2018.10.066
, Parametric Analysis and Optimization of a Solar Driven Trigeneration System Based on ORC and Absorption Heat Pump ,J. Clean. Prod. , Vol. 161 ,pp 493-509 , 2017, https://doi.org/https://doi.org/10.1016/j.jclepro.2017.05.159
, Dynamic Modeling of a Solar ORC With Compound Parabolic Collectors: Annual Production and Comparison With Steady-State Simulation ,Energy Convers. Manag. , Vol. 148 ,pp 708-723 , 2017, https://doi.org/https://doi.org/10.1016/j.enconman.2017.06.025
, Development and Experimental Characterization of a Small Scale Solar Powered Organic Rankine Cycle (ORC) ,Energy Procedia , Vol. 101 ,pp 504-511 , 2016, https://doi.org/https://doi.org/10.1016/j.egypro.2016.11.064
, Techno-Economic Comparison of Solar Organic Rankine Cycle (ORC) and Photovoltaic (PV) Systems With Energy Storage ,Renew. Energy , Vol. 113 ,pp 1250-1260 , 2017, https://doi.org/https://doi.org/10.1016/j.renene.2017.06.107
, Investigation of a Hybrid ORC Driven by Waste Heat and Solar Energy ,Energy Convers. Manag. , Vol. 156 ,pp 427-439 , 2018, https://doi.org/https://doi.org/10.1016/j.enconman.2017.11.058
, Energy and Exergy Analyses of Various Typical Solar Energy Applications: A Comprehensive Review ,Renew. Sustain. Energy Rev. , Vol. 82 (Part 1),pp 1576-1601 , 2018, https://doi.org/https://doi.org/10.1016/j.rser.2017.07.003
, Transient Simulation of a Solar Heating System for a Small-Scale Ethanol-Water Distillation Plant: Thermal, Environmental and Economic Performance ,Energy Convers. Manag. , Vol. 134 ,pp 347-360 , 2017, https://doi.org/https://doi.org/10.1016/j.enconman.2016.12.041
, The Potential of Solar Heat for Industrial Processes in Germany ,Renew. Sustain. Energy Rev. , Vol. 16 (7),pp 5121-5130 , 2012, https://doi.org/https://doi.org/10.1016/j.rser.2012.04.032
, Review of Passive PCM Latent Heat Thermal Energy Storage Systems Towards Buildings Energy Efficiency ,Energy and Buildings , Vol. 59 ,pp 82-103 , 2013, https://doi.org/https://doi.org/10.1016/j.enbuild.2012.12.042
, Solar energy use in district heating systems. A case study in Latvia ,Energy , (137),pp 586–94 , 2017, https://doi.org/https://doi.org/10.1016/j.energy.2017.04.151
, Classification of Industrial Heat Consumers for Integration of Solar Heat ,Energy Procedia , Vol. 91 ,pp 650-660 , 2016, https://doi.org/https://doi.org/10.1016/j.egypro.2016.06.225
, Modeling and Design of Solar Heat Integration in Process Industries With Heat Storage ,J. Clean. Prod. , Vol. 170 ,pp 522-534 , 2018, https://doi.org/https://doi.org/10.1016/j.jclepro.2017.09.183
, A New Method for Optimization of Solar Heat Integration and Solar Fraction Targeting in Low Temperature Process Industries ,Energy , Vol. 90 (Part 2),pp 1674-1681 , 2015, https://doi.org/https://doi.org/10.1016/j.energy.2015.06.128
, Process Integration in Batch Processes ,IChemE Symp. Ser. , Vol. 109 ,pp 221-237 , 1988
, , Heat Exchanger Network Synthesis: Process Optimization by Energy and Resource Analysis , Vol. 1-2 , 1995
, , Pinch Analysis and Process Integration (A User Guide on Process Integration for the Efficient Use of Energy) , 2007
, Methods Optimisation, Process Integration and Modelling for Energy Saving and Pollution Reduction ,Energy , Vol. 146 ,pp 1-3 , 2018, https://doi.org/https://doi.org/10.1016/j.energy.2018.01.122
, Extending Total Site Methodology to Address Varying Energy Supply and Demand ,Handbook of Process Integration ,pp 226-261 , 2013, https://doi.org/https://doi.org/10.1533/9780857097255.2.226
, Integration and Management of Renewables into Total Sites With Variable Supply and Demand ,Comput. Chem. Eng. , Vol. 35 (9),pp 1815-1826 , 2011, https://doi.org/https://doi.org/10.1016/j.compchemeng.2011.02.009
, Algorithmic Targeting for Total Site Heat Integration With Variable Energy Supply/Demand ,Appl. Therm. Eng. , Vol. 70 (2),pp 1073-1083 , 2014, https://doi.org/https://doi.org/10.1016/j.applthermaleng.2014.03.014
, Integration of Solar Thermal Energy into Processes With Heat Demand ,Clean Technol. Environ. Policy , Vol. 14 (3),pp 453-463 , 2012, https://doi.org/https://doi.org/10.1007/s10098-012-0457-6
, Integration of Solar Thermal for Improved Energy Efficiency in Low-Temperature-Pinch Industrial Processes ,Energy , Vol. 35 (5),pp 1867-1873 , 2010, https://doi.org/https://doi.org/10.1016/j.energy.2009.06.039
, Integration of Industrial Solar and Gaseous Waste Heat into Heat Recovery Loops Using Constant and Variable Temperature Storage ,Energy , Vol. 75 ,pp 53-67 , 2014, https://doi.org/https://doi.org/10.1016/j.energy.2014.01.103
, Process Integration Techniques for Cogeneration and Trigeneration Systems ,Handbook of Process Integration ,pp 484-504 , 2013
, Application of Process Integration to the Synthesis of Heat and Power Utility Systems Including Combined Heat and Power (CHP) and Industrial Heat Pumps ,Handbook of Process Integration ,pp 168-200 , 2013
, Strategy to Synthesize Integrated Solar Energy Coproduction Processes With Optimal Process Intensification. Case Study: Efficient Solar Thermal Hydrogen Production ,Comput. Chem. Eng. , Vol. 105 ,pp 328-347 , 2017, https://doi.org/https://doi.org/10.1016/j.compchemeng.2017.01.038
, Design and Optimization of a Novel System for Trigeneration ,Energy , Vol. 168 ,pp 247-260 , 2019, https://doi.org/https://doi.org/10.1016/j.energy.2018.11.086
, Energetic and Exergetic Analysis of a Solar-Assisted Combined Power and Cooling (SCPC) System With Two Different Cooling Temperature Levels ,Energy Convers. Manag. , Vol. 182 ,pp 497-507 , 2019, https://doi.org/https://doi.org/10.1016/j.enconman.2018.12.069
, Modelling and Analysis of a Hybrid Solar Concentrating-Waste Incineration Power Plant ,J. Clean. Prod. , Vol. 216 ,pp 570-584 , 2019, https://doi.org/https://doi.org/10.1016/j.jclepro.2018.12.055
, Visions for Small-Scale Renewable Energy Production on Finnish Farms – A Delphi Study on the Opportunities for New Business ,Energy Policy , Vol. 129 ,pp 939-948 , 2019, https://doi.org/https://doi.org/10.1016/j.enpol.2019.03.004
, Recent Advancements in Sorption Technology for Solar Thermal Energy Storage Applications ,Solar Energy , Vol. 192 ,pp 69-105 , 2018, https://doi.org/https://doi.org/10.1016/j.solener.2018.06.102
, Description and Evaluation of the New 1,000 kWel Organic Rankine Cycle Process Integrated in the Biomass CHP Plant in Lienz, Austria ,Euroheat & Power , Vol. 10 ,pp 1-17 , 2002
, Solar Energy: Markets, Economics and Policies ,Renew. Sustain. Energy Rev. , Vol. 16 (1),pp 449-465 , 2012, https://doi.org/https://doi.org/10.1016/j.rser.2011.08.009
,